FY2019 Annual Report
Developmental Neurobiology Unit
Professor Ichiro Masai
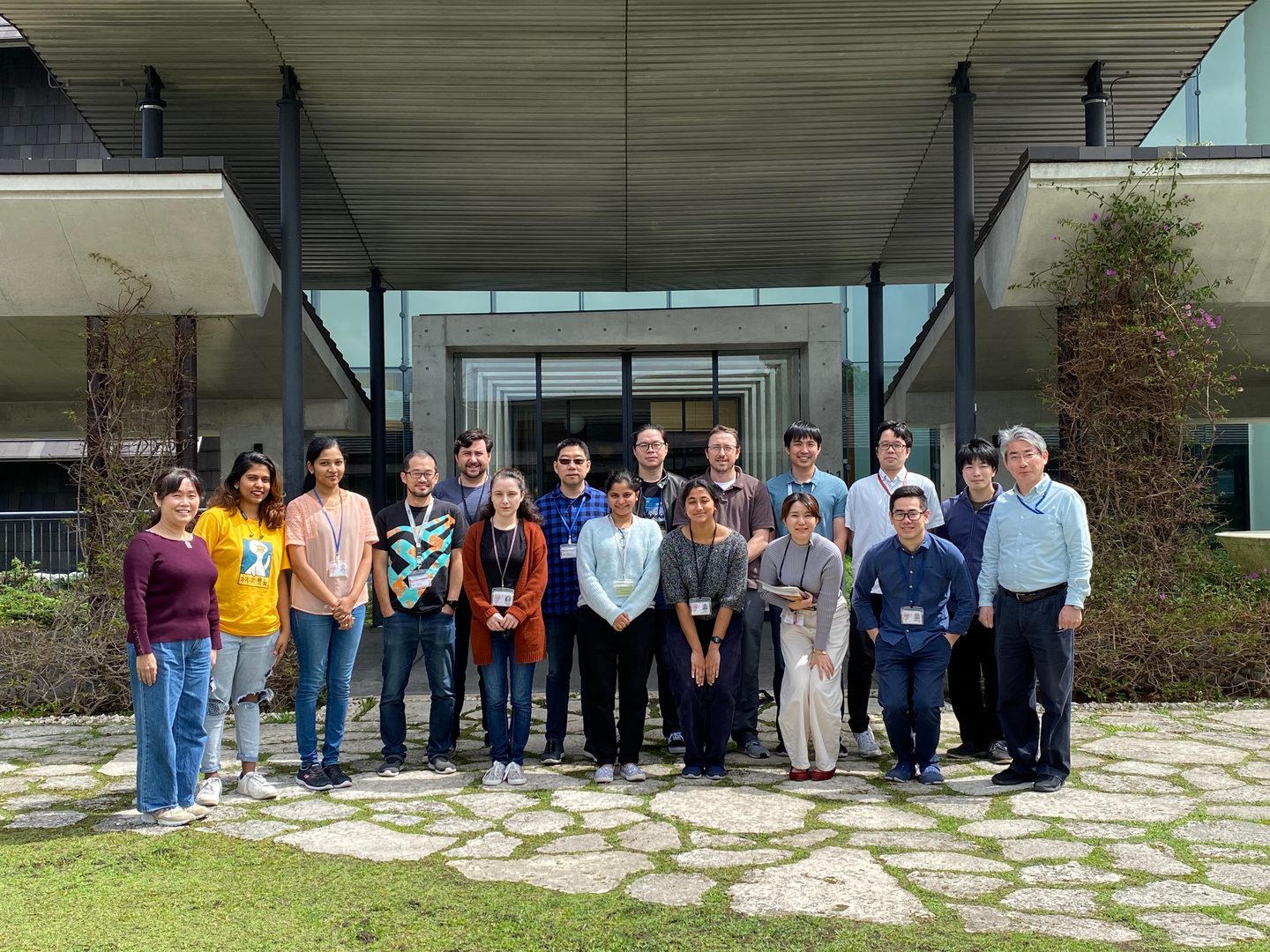
Abstract
Developmental neurobiology unit uses zebrafish as an animal model and will elucidate genetic program that regulate eye development. Specific research projects are to elucidate:
- Mechanisms that regulate retinal cell differentiation and neural circuit formation
- Mechanisms that regulate photoreceptor degeneration
- Mechanisms that regulate lens fiber differentiation
During vertebrate development, the retina is originally derived from anterior neural plate. In this region, six major classes of retinal neurons differentiate and form neural circuits responsible for vision. Thus, the retina provides a good model for studying cell differentiation and neural circuit formation in the developing brain. First, we will investigate mechanisms that regulate cell differentiation and neural circuit formation. Second, we will investigate mechanisms that regulate photoreceptor degeneration. Photoreceptor degeneration is an important topic for medical research, because more than 300 genetic mutations are associated with photoreceptor degeneration in humans. We focus on zebrafish mutants, in which photoreceptor degeneration is caused by defects in protein synthesis in ER, protein transport from ER to the apical photoreceptive membrane region, and phototransduction. Through these mutant analyses, we will determine how photoreceptors monitor abnormalities in cellular functions and trigger apoptosis. We will also investigate the role of microglia in photoreceptor degeneration. Third, we will investigate lens development. The lens is an intraocular organ that focuses visual image on retinal photoreceptors. Lenses consist of two cell-types: lens epithelial cells and lens fiber cells. Lens epithelial cells differentiate into lens fiber cells, each of which are integrated in a stereotyped geometric pattern to form spherical lens fiber core. We will investigate mechanisms that regulate lens fiber differentiation. Through these projects, we will establish key concepts that govern development of multicellular organisms and also contribute to our understanding of pathological processes of human retinal diseases.
1. Staff
- Dr. Yuko Nishiwaki, Group leader
- Dr. Yuki Sugiyama, Staff scientist
- Dr. Yuki Takeuchi, Staff scientist
- Dr. Wei-Chieh Chiang, Staff scientist (1 June 2019–)
- Dr. Luis Carretio, Postdoctral scholar (HFSP) (16 July 2019–)
- Ms. Nishtha Ranawat, phD student
- Ms. Manana Kutsia, phD student
- Ms. Mai Omar Abdulrahman Ahmad, phD student
- Ms. Swathy Babu, phD student
- Mr. Bedish Chatterjee, phD student
- Mr. Hung-Ju Chiang, phD student
- Mr. Dongpeng Hu, phD student (1 Sept 2019 –)
- Ms. Darshini Ravishanker (1 Sept 2019–)
- Mr. Yutaka Kojima, Technician
- Mr. Kevin Jeff Liner, Technician
- Ms. Kimberlie Ward, Technician (–12 July 2019)
- Dr. Tetsuya Harakuni, Technician
- Dr. Mamoru Fujiwara, Technician (Tempo staff)
- Mr. Takuya Kamichika (Tempo staff, HFSP) (16 Sept 2019–)
- Ms. Versha Venkatesha Murthy
- Ms. Moe Inafuku, Laboratory Assistant
- Ms. Chitose Mizuta, Laboratory Assistant
- Ms. Rui Inoue, Laboratory Assistant
- Ms. Fujino Ishibashi (18 Nov 2019–)
- Ms. Satsuki Asato, Laboratory Assistant (–31 Dec 2019)
- Ms. Madoka Makiya, Research Assistant (Tempo staff)
- Ms. Maki Ishikawa, Research Assistant (Tempo staff) (–30 Nov 2019)
- Ms. Miki Kitamura (9 March 2020–)
- Ms. Ayako Gima, Research Administrator/Secretary (–30 Nov 2019)
- Ms. Mizuki Otake, Research Administrator/Secretary (1 Oct 2019–)
2. Collaborations
2.1 The Title or Name or Topic of the Collaboration
- Theme: In vivo functional analysis of hypoxia response genes using the zebrafish retina
- Type of collaboration: Joint research agreement
- Researchers: Dr. Ichiro Masai (Developmental neurobiology unit, OIST), Dr. Masayuki Matsushita (Department of Medicine, Ryukyu University)
2.2 The Title or Name or Topic of the Collaboration
- Theme: Role of zebrafish BANP protein in tumor suppression of melanoma
- Type of collaboration: Joint research agreement
- Researchers: Dr. Ichiro Masai (Developmental neurobiology unit, OIST), Dr. Yutaka Kikuchi (Department of Biological Science, Hiroshima University)
3. Activities and Findings
3.1 Mechanism underlying photoreceoptor degeneration
Apoptosis is observed in developing tissues and is believed to remove abnormal cells. Although apoptosis is important for establishment of proper neural circuits by eliminating abnormally differentiated neurons, it is unclear how differentiating cells monitor their own abnormality, and how the threshold at which apoptosis is induced is determined. Photoreceptors provide a useful model for studying such a surveillance mechanism of neuronal development and homeostasis, because there are many hereditary retinal diseases in humans associated with photoreceptor degeneration. Although 271 genes linked to hereditary retinal diseases have already been identified, these genes encode diverse functions, including phototransduction, retinol metabolism, and intracellular protein transport. To answer how photoreceptors monitor their differentiation status and homeostasis, and what kinds of molecular network determine the choice between cell survival and cell death in photoreceptors, we have investigated zebrafish mutants in which photoreceptor degeneration is triggered by defects in phototransduction, intracellular vesicular transport, and intraflagellar transport via the primary cilium. We also investigated the role of brain-resident immune cells, microglia, in photoreceptor degeneration.
3.1.1 Mechanism of photoreceptor degeneration in response to vesicular fusion defects
Intracellular protein transport is mediated by transport vesicles and its defects are often linked to photoreceptor degeneration in humans. However, it is uncertain how vesicular transport defects cause photoreceptor degeneration. We isolated zebrafish β-soluble N-ethylmaleimide-sensitive factor attachment protein (β-SNAP) mutants, in which photoreceptors differentiate, but undergo apoptosis prior to maturation (Nishiwaki et al., 2013) (Fig. 1A). β-SNAP cooperates with N-ethylmaleimide-sensitive factor (NSF) to promote recycling of SNAP receptors (SNARE), a key component of vesicular fusion machinery, by disassembling the cis-SNARE complex generated in the vesicular fusion process (Jahn and Scheller, 2006). Because it is likely that all combinations of cis-SNARE complexes fail to be disassembled in β-SNAP mutants, the mutant provides a good model for studying how vesicular transport defects cause apoptosis in photoreceptors. We found that photoreceptor apoptosis in β-SNAP mutants depends on one of t-SNARE proteins, BNip1. BNip1 normally regulates retrograde transport from Golgi to ER as a t-SNARE component of the syntaxin-18 complex. Interestingly, BNip1 has the BH3 domain, which activates apoptosis via modulation of apoptotic regulators Bax and Bcl2. We found that loss of β-SNAP induces accumulation of syntaxin-18 cis-SNARE complex, in which the BNip1 BH3 domain is activated to induce apoptosis. Thus, the syntaxin-18 cis-SNARE complex functions as an alarm factor that monitors vesicular fusion competence, and BNip1 transforms vesicular fusion defects into photoreceptor apoptosis (Fig. 1B) (Nishiwaki et al., 2013). To our knowledge, BNip1 is the first molecule that directly links vesicular fusion defects and apoptosis.
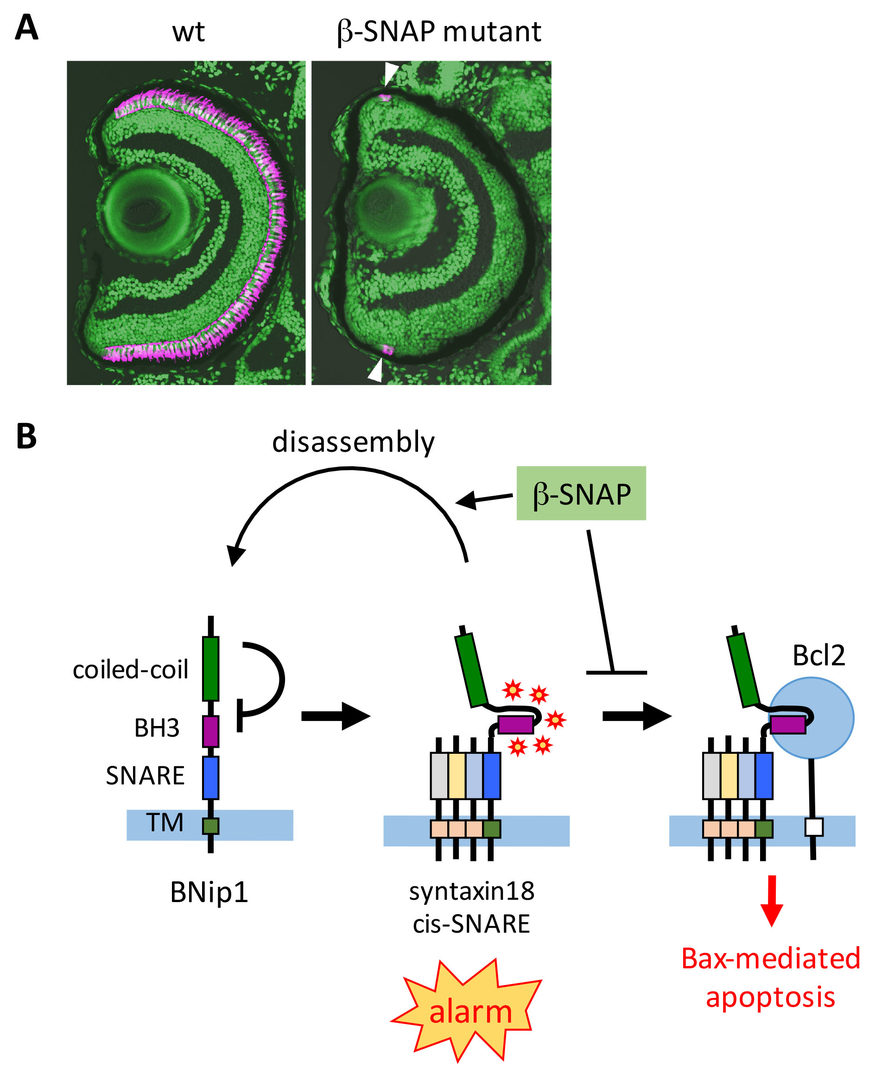
Fig. 1: Surveillance mechanism that monitors vesicular fusion defects in photoreceptor. (A) Wild-type and β-SNAP mutant retina. Photoreceptors are labeled in magenta. In b-SNAP mutants, photoreceptors once differentiate (arrowheads) quickly undergo degeneration. (B) Syntaxin-18 cis-SNARE complex functions as an alarm of vesicular fusion defects. β-SNAP normally disassemble the cis-SNARE complex for SNARE recycle. In β-SNAP mutants, syntaxin-18 cis-SNARE complex is accumulated, and then the BNip1 BH3 domain is activated to induce Bax-dependent apoptosis. This system converts vesicular fusion defects into apoptotic program.
Next, we investigated a physiological role of BNip1-dependent apoptosis. In zebrafish β-SNAP mutants, photoreceptors undergo apoptosis mainly in a small time window of developmental stages, 2 - 4 dpf in which apical photoreceptive membrane structure, called the outer segment (OS) rapidly grows. Since protein synthesis and transport to the OS are highly active in this period, photoreceptor apoptosis in β-SNAP mutants correlates with high levels of protein transport. We examined a critical period of β-SNAP for photoreceptor maintenance by overexpressing β-SNAP under the control of heat shock promoter in β-SNAP mutants (Fig. 2A). We found that overexpression of β-SNAP in 2-5 dpf is enough to prevent photoreceptor apoptosis in zebrafish β-SNAP mutants at least until 21 dpf (Fig. 2B, C). These data raise the possibility that BNip1-mediated apoptosis links to excessive activation of vesicular transport associated with rapid growth of the OS.
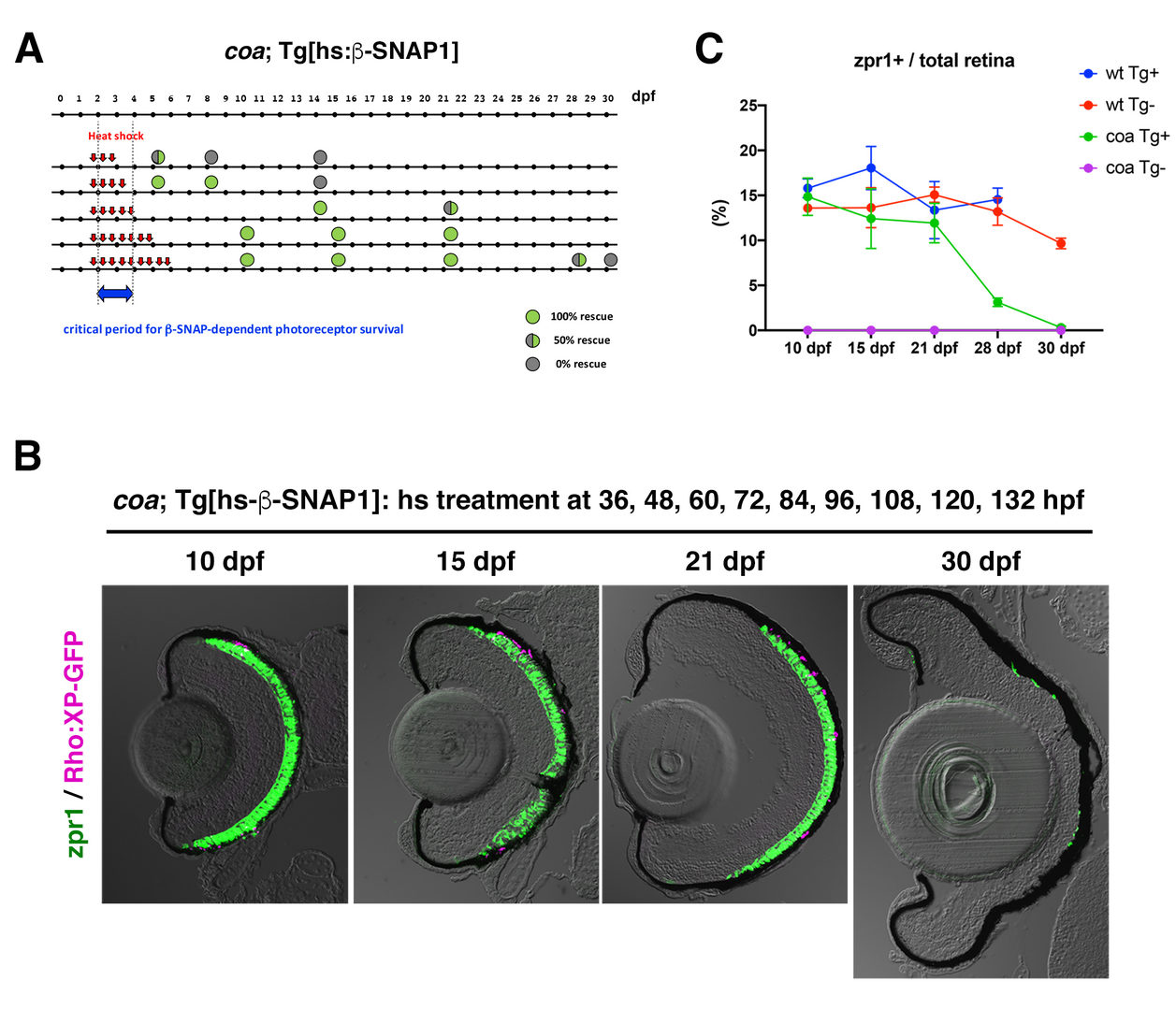
(A) Experimental design and results of heat shock promoter-driven β-SNAP1 overexpression in b-SNAP1 mutants, coa. Zebrafish transgenic line, Tg[hs: β-SNAP1], were treated with heat treatment at different time points with a 12 hours interval in the period from 36 to 132 hpf. Red arrows indicate one hour-pulsed heat shock treatment at 37oC. Green or grey colored circle indicate the rescue results and its position indicates stage when embryos or larvae were fixed with PFA for analysis. Blue arrow indicates the critical period for β-SNAP1-dependent photoreceptor survival.
(B) Retinas of coa; Tg[rho:XP:GFP]; Tg[hs: β-SNAP1] embryos or larvae, which were treated with heat shock nine times at 36, 48, 60, 72, 84, 96, 108, 120, and 132 hpf. Photoreceptors and their OS were visualized by labeling with zpr1 antibody (green) and GFP signals from Tg[rho:XP:GFP] (magenta), respectively. Photoreceptors were maintained at 10, 14, 21 dpf, but degenerated at 30 dpf.
(C) Time line of percentage of zpr1-positive area relative to total retinal area at 10, 15, 21, 28 and 30 dpf. Average percentage of wild-type and coa mutant embryos with and without Tg[hs: β-SNAP1]. Photoreceptors survived in coa mutant embryos with Tg[hs: β-SNAP1] by 21 dpf, degenerated at 28 dpf and completely eliminated at 30 dpf (green line).
Consistently, knockdown of IFT88 and Kif3b, which inhibits transport to the OS, rescued photoreceptor apoptosis in β-SNAP mutants. The treatment of rapamycin, which inhibits protein synthesis through mTOR pathway, also rescued photoreceptor apoptosis in β-SNAP mutants. Importantly, the ER stress response is not activated 2-3 dpf in β-SNAP mutants. Taken together, these data support our model in which BNip1 performs risk assessment that detects excessive vesicular transport in photoreceptors (Fig. 3). In general, deletion of β-SNAP activity compromises recycling of SNARE proteins, which eventually arrests all intracellular protein transport from ER to plasma membrane. In this case, it is likely that the ER stress response is activated to induce apoptosis. However, our study of zebrafish β-SNAP mutants indicates that prior to activation of the ER stress response, BNip1 detects vesicular fusion defects during a rapid OS growth period. Thus, photoreceptors use two different surveillance mechanisms, BNip1 and ER stress response, to monitor the upper and lower ranges of vesicular transport during development.
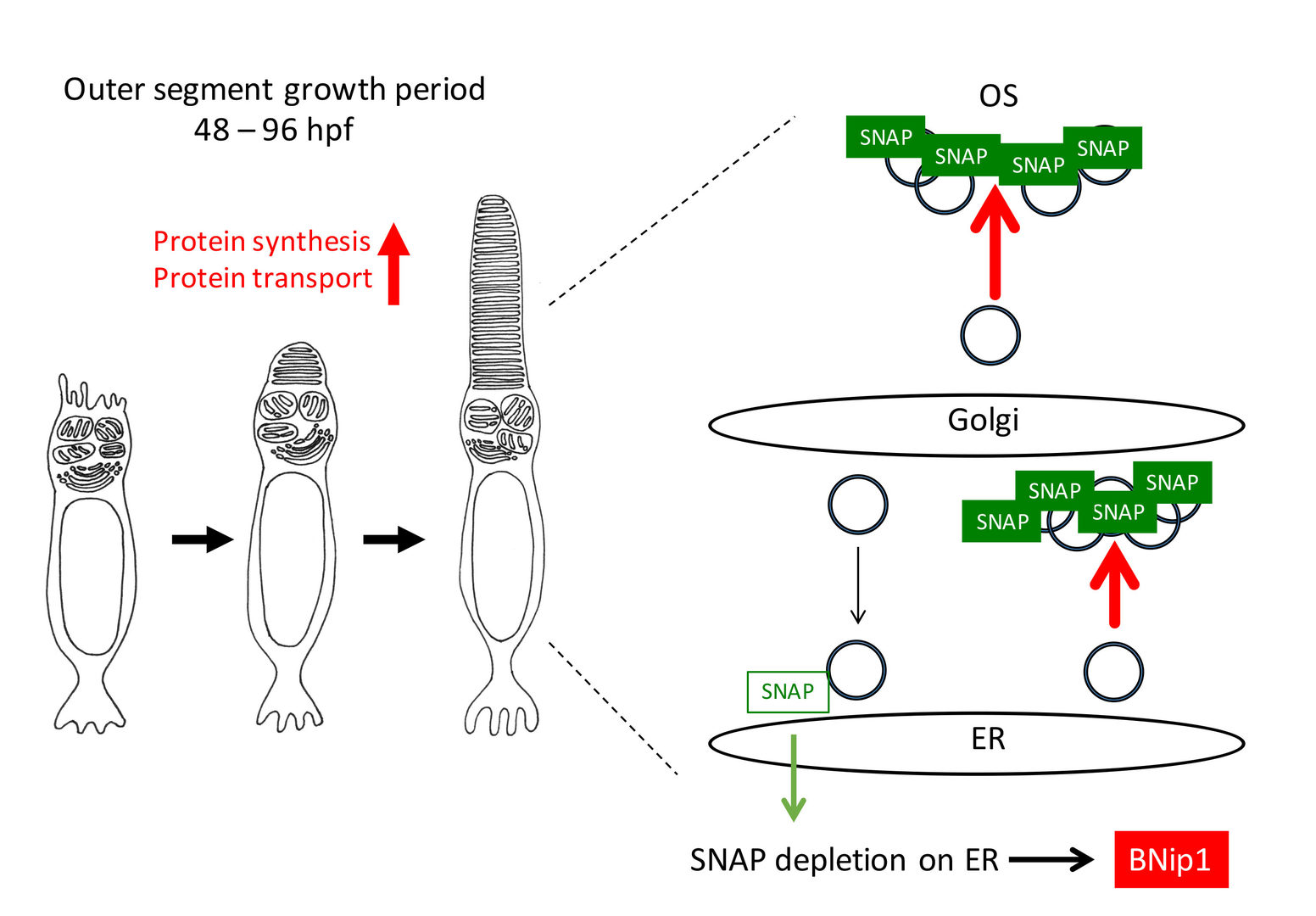
3.1.2 Mechanism of neuronal regeneration in response to photoreceptor degeneration
Unlike mammals, zebrafish have the capacity to regenerate neurons in response to retinal damage. Müller cells are the only glial cell type in the retina. After retinal damages including photoreceptor degeneration, Müller cells are reprogrammed to a retinal progenitor cell-like state and generate neuronal progenitor cells, which subsequently differentiate into all the retinal cell types and rebuild the lost neural circuit. In FY2019, we studied the regenerative response in the zebrafish gold rush (gosh) mutants. Zebrafish gosh mutants show progressive degeneration of both rod and cone photoreceptors at embryonic stages (Fig. 4A), but later Müller cells start regeneration program that generates rods, resulting in cone-specific elimination at the adult stage (Fig. 4B). We found that gosh mutant gene encodesarylhydrocarbon receptor interacting protein like 1b (aipl1b) (Iribarne et al., 2017). There are two aipl1 genes in zebrafish, aipl1a and aipl1b, whose mRNAs are expressed exclusively in rods and cones, respectively (Fig. 4C). In human, genetic mutations of AIPL1 gene induces rod and cone dystrophy, which is known as Leber congenital amaurosis 4 (LCA4). In mice, AIPL1 is required for protein stability of cGMP phosphodiesterase 6 (PDE6), which regulates phototransduction in vertebrate photoreceptors (Fig. 4D). We showed that cone-specific subunit of PDE6, namely PDE6c, is unstable in gosh mutants (Fig. 4E). Indeed, since both gosh and pde6c mutants share phototransduction defects, photoreceptor degeneration profile are very similar between them (Nishiwaki et al., 2008) (Fig. 4B). So, the gosh mutants provide a good model to study how photoreceptors undergo degeneration in response to phototransduction defects, and how Müller cells are reprogrammed to initiate neuronal regeneration in response to photoreceptor degeneration.
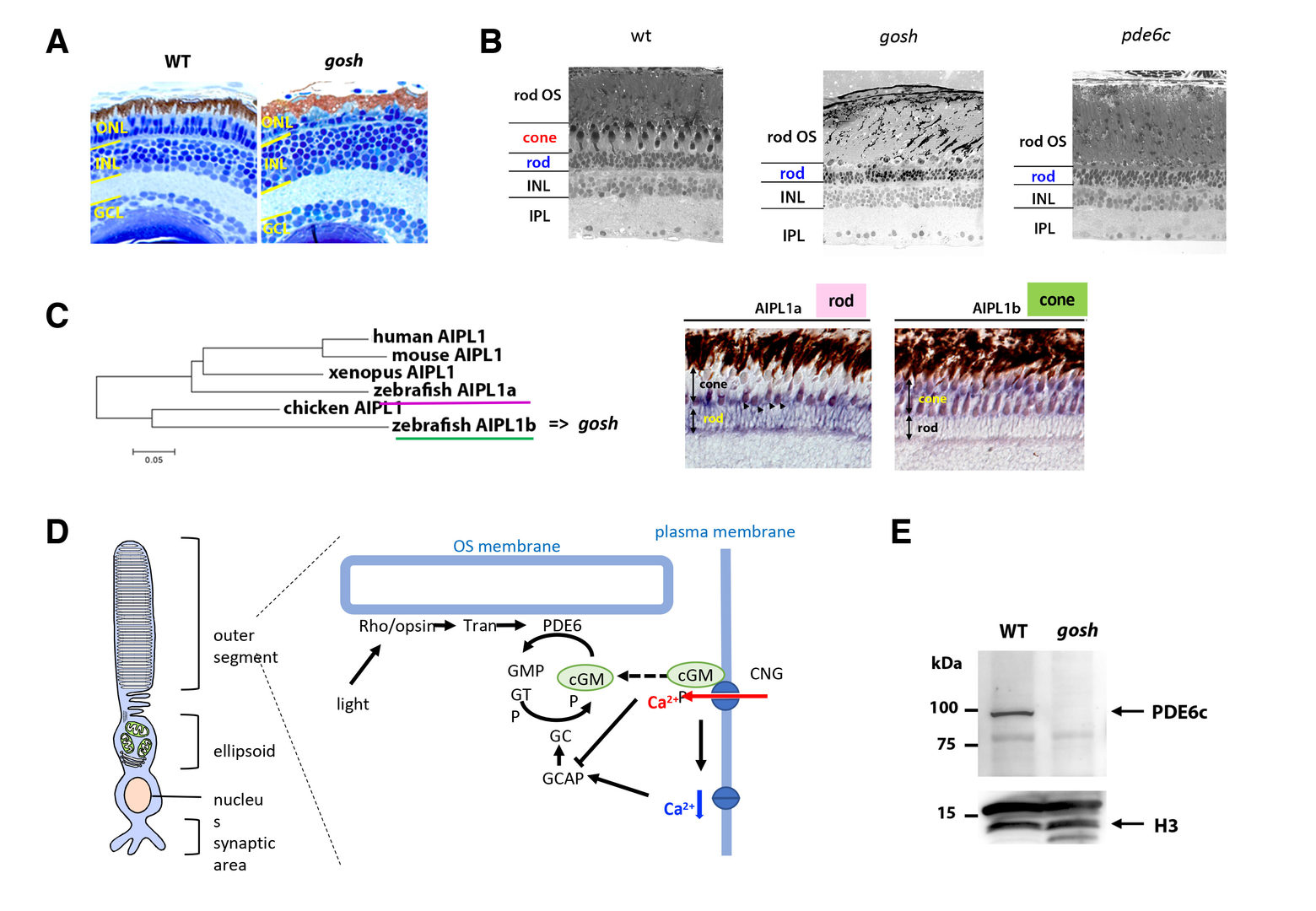
Wild-type and gosh mutant retinas at 7 dpf. (B) Wild-type, gosh mutant, and pde6c mutant retinas at adult stage (12 wpf). (C) Phylogenetic tree of AIPL1 proteins of humans, mice, chicks, Xenopus, and zebrafish Aipl1a and Aipl1b. (D) Expression of aipl1a and aipl1b mRNAs in wild-type adult retina. aipl1b mRNA is expressed exclusively in cones, while aipl1a mRNA is expressed in rods. (D) Schematic drawing of phototransduction cascade. PDE6 mediates phototransduction by hydrolysis of the second messenger cGMP, which opens CNG channel in photoreceptors. (E) Western blot of wild-type and gosh mutant heads with anti-Pde6c antibody. A band of approximately 100 kDa was detected in wild type, but disappeared in the gosh mutant. Histone H3 is as a loading control.
In FY2019, we investigated developmental profile of photoreceptor degeneration and Müller cell-mediated regeneration in gosh mutants (Fig. 5A). In gosh mutants, both cones and rods degenerate by 3 weeks post-fertilization (wpf) (Fig. 5B). Müller glia do not exhibit a regenerative response by 3 wpf; however, they do present non-proliferative gliosis (Fig. 5C). Only at 5 wpf, proliferation of Müller cells and rod precursor cells is activated (Fig. 5D). Rods start to recover at 5 wpf and by 12 wpf they reach a level of recovery comparable to wild type, but cones remain absent in the adult stage. TNF-alpha was detected in degenerating cones at 5-7 wpf (Fig. 5E) and in Müller glia at 7 wpf in goshmutants (Fig. 5F). At 5 wpf, proliferating Müller glia express Sox2, followed by Pax6 expression in neuronal progenitor cells, confirming that the neuronal regeneration program is activated in gosh mutants after 5 wpf. Although acute light-induced damage did not activate proliferation of Müller glia, TNF- injection caused Müller glia to commence a proliferative response at 3 wpf in gosh mutants. These results suggest that Müller glia transition from non-proliferative gliosis to a regenerative state in gosh mutants, and that ectopic introduction of TNF-alpha promotes this Müller cell transition even at 3 wpf (Iribarne et al., 2019). Thus, zebrafish gosh mutants provide a useful model to investigate mechanisms underlying retinal regeneration in a chronic photoreceptor degeneration model.
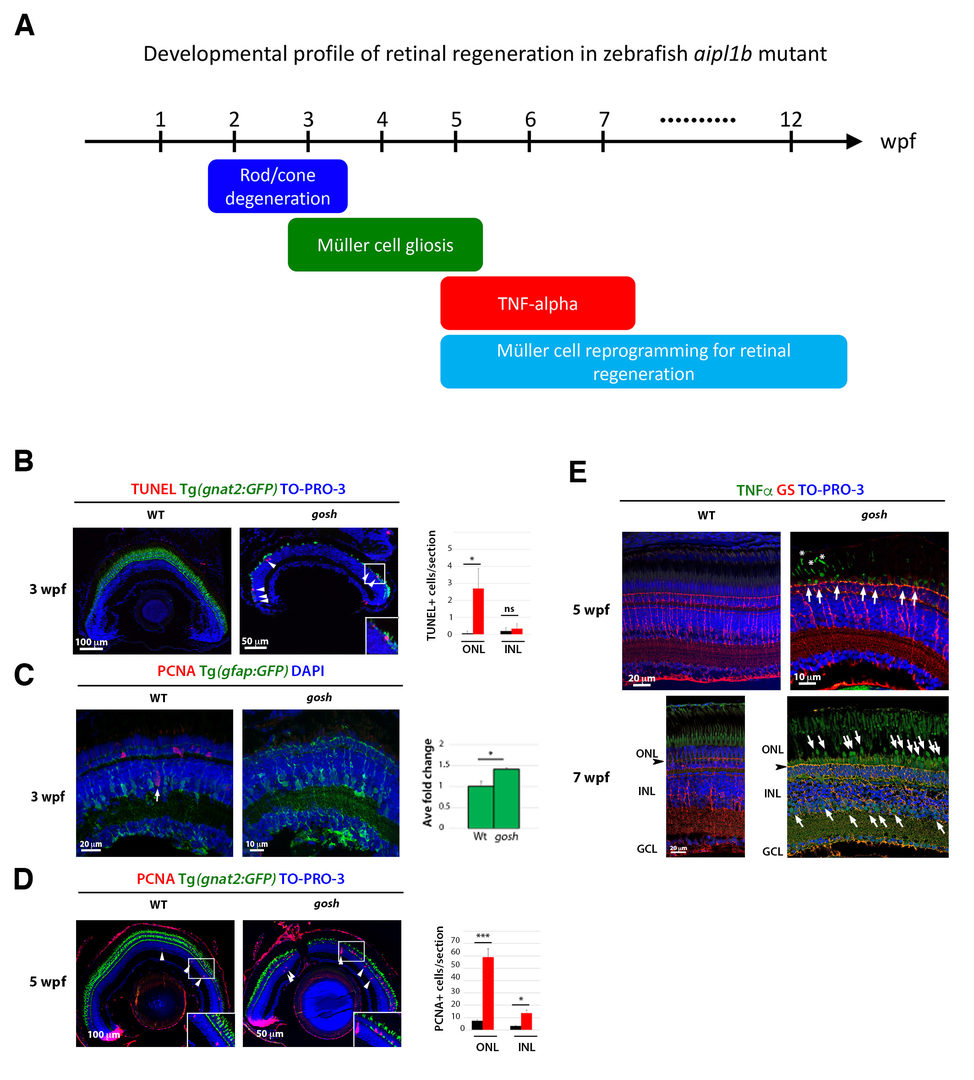
(A) Schematic drawing of developmental profile of retinal degeneration and regeneration in zebrafish mutants. Rod and cone degeneration mainly occurs in 2-3 wpf. Müller cells show gliosis in 3-5 wpf. TNF-alpha starts to be expressed in dying photoreceptor at 5 wpf and also in Müller cells at 7 dpf. Müller cells are reprogramming to enter cell-cycle progression and generate neuronal progenitor cells after 5 wpf. (B) TUNEL (red) of wild-type and gosh mutant retinas at 3 wpf. gnat2:GFP indicates cone photoreceptors in green, and TOPRO3 indicates nuclei in blue. TUNEL signals are detected in ONL in gosh mutants. (C) GFAP:GFP expression (green) in wild-type and gosh mutant retinas at 3 wpf. Proliferating cells are labeled with PCNA (red). In gosh mutants, GFAP:GFP expression is upregulated in Müller cells, which show a great number of cell processes and also PCNA-negative, suggesting non-proliferative gliosis. (D) PCNA expression in wild-type and gosh mutant retinas at 5 wpf. gnat2:GFP indicates cone photoreceptors in green, and TOPRO3 indicates nuclei in blue. PCNA-positive ONL and INL cells are drastically increased in gosh mutants. (E) TNF-alpha expression in wild-type and gosh mutant retinas at 5 and 7 wpf. Müller cells are labeled with glutamine synthetase (GS) (red). In gosh mutants, TNF-alpha expression is observed in degenerating cones at 3 and 7 wpf (arrows), and in Müller cells of INL at 7 wpf (arrowhead).
3.2 The role of microglia in retinal development and degeneration
Microglia are brain-resident immune cells, originally derived from mesoderm-derivative tissue or the hematopoietic stem cell-lineage, that migrate into brain, and patrol within the brain throughout life. Microglia are thought to eliminate dead or dying neurons to prevent inflammation. Interestingly, microglia-mediated inflammation is required for neuronal regeneration in response to traumatic brain injury in zebrafish (Kyritsis et al., 2012), suggesting more dynamic roles of microglia in neuronal damage. Furthermore, surprisingly, it was reported that microglia facilitate rod cell death in rod-PDE6 mutant mice, which contrasts with our previous view that microglia promote neuronal protection (Zhao et al., 2015). Thus, it is important to understand the role of microglia in photoreceptor degeneration.
We examined normal developmental profiles of microglia and their colonization mechanism to the zebrafish retina. It was reported that microglia are initially generated in lateral plate mesoderm, but that they move to yolk, and enter the brain, including the eyes during zebrafish development (Herbomel et al., 2001). However, it is unknown what kinds of guidance cues enable microglia to move from yolk into developing retina, how microglia are integrated into retinal neural circuits, and also how much cell proliferation contributes to the number of microglia colonizing the retina during development. To answer these questions, we conducted time-lapse observation using zebrafish mpeg1:GFP or mfap4:tdTomato transgenic lines, which specifically visualizes microglia in zebrafish (Ellett et al., 2011; Walton et al., 2015). We found that microglia progressively enter the optic cup from 24 to 54 hpf through the ventral optic fissure. After entry into the optic cup, microglia did not proliferate, suggesting that increased numbers of microglia largely depend on migration from the optic cup. We are now investigating molecular mechanism that guides microglia into optic cup during development.
3.3 Mechanism underlying lens development
The lens is an intraocular organ that focuses visual images on retinal photoreceptors. The lens consists of two cell types: lens epithelial cells and lens fiber cells. Lens epithelium convers the anterior half of the lens fiber core (Lovicu and McAvoy, 2005; Mochizuki and Masai, 2014). At the lens equator, epithelial cells start to differentiate into lens fiber cells, which elongate and pile up to cover the old lens fiber core. Thus, the lens provides a good model for studying spatiotemporal coordination of cell differentiation and morphogenesis (Fig. 6). In vertebrate lens, FGF is secreted from the retina and is believed to form a low-high gradient along the anterior-posterior axis of the lens. It was reported that FGF promotes cell proliferation of lens epithelial cells at low doses and lens fiber differentiation at high doses (McAvoy et al., 2017) (Fig. 6). These observations raised the “FGF gradient hypothesis”, in which FGF regulates multiple steps of lens fiber differentiation: low doses of FGF promote lens epithelial cell proliferation, whereas high doses of FGF induce lens fiber cell differentiation. Furthermore, previous studies revealed a network of transcription factors that coordinate lens epithelial cell proliferation in the anterior lens and lens fiber differentiation in the posterior lens (Fig. 6). These transcription factors are classified into two groups. In the anterior lens epithelium, Pax6 activates Mab21l1 and Foxe3 in concert with Pitx3 and Msx2, resulting in maintenance of E-cadherin expression and a proliferative state of lens epithelial cells. In the posterior lens fiber region, Prox1 activates cell-cycle inhibitor, p57kip2, to promote cell-cycle exit of lens epithelial cells at the equator. In addition, c-Maf and Sox1 activate expression of b- and g-cyrstallin to promote lens fiber maturation. These two groups of transcription factors mutually repress each other to maintain the boundary between the lens epithelium and lens fiber areas (Fig. 6). However, the mechanism that spatially regulates lens epithelial proliferation and lens fiber differentiation is still not fully understood.
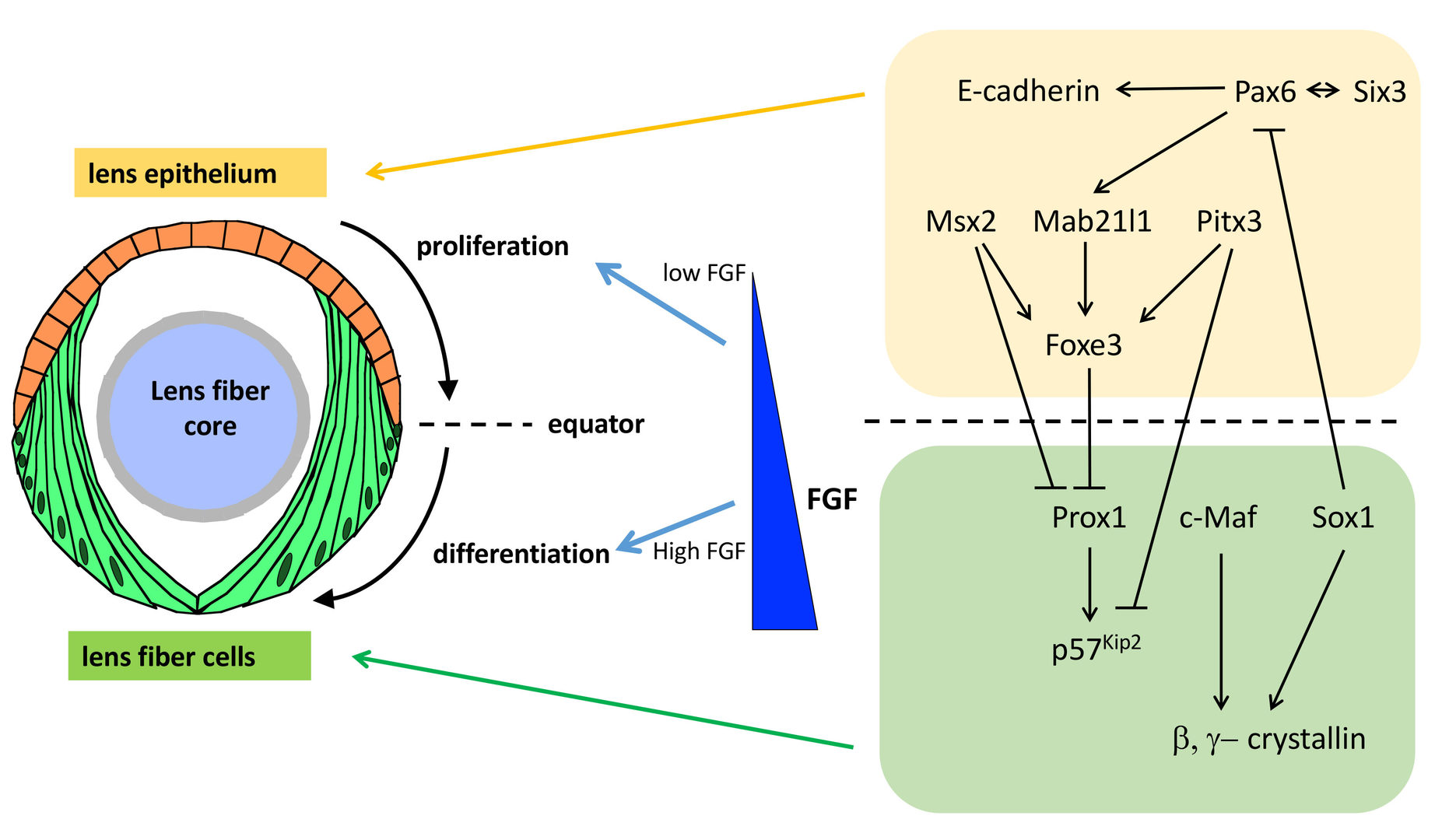
3.3.1 Mechanisms that regulates equator-specific onset of lens fiber differentiation
In order to elucidate the mechanism underlying equator-specific onset of lens fiber differentiation, we screened zebrafish mutants. We identified zebrafish vps45 mutants, in which lens epithelial cells undergo ectopic lens fiber differentiation without passing through the equator (Fig. 7A) (Mochizuki et al., 2018). VPS45 regulates vesicular transport from early endosomes to late/recycling endosomes, suggesting that endolytic trafficking mechanism suppresses ectopic lens fiber differentiation in lens epithelium. Interestingly, this ectopic lens fiber differentiation is mediated by activation of TGF-bsignaling and inhibition by Wnt signaling pathways (Fig. 7B). Our findings indicate a novel FGF-independent mechanism that suppresses lens fiber differentiation in anterior lens epithelium and ensures equator-specific commencement of lens fiber differentiation.
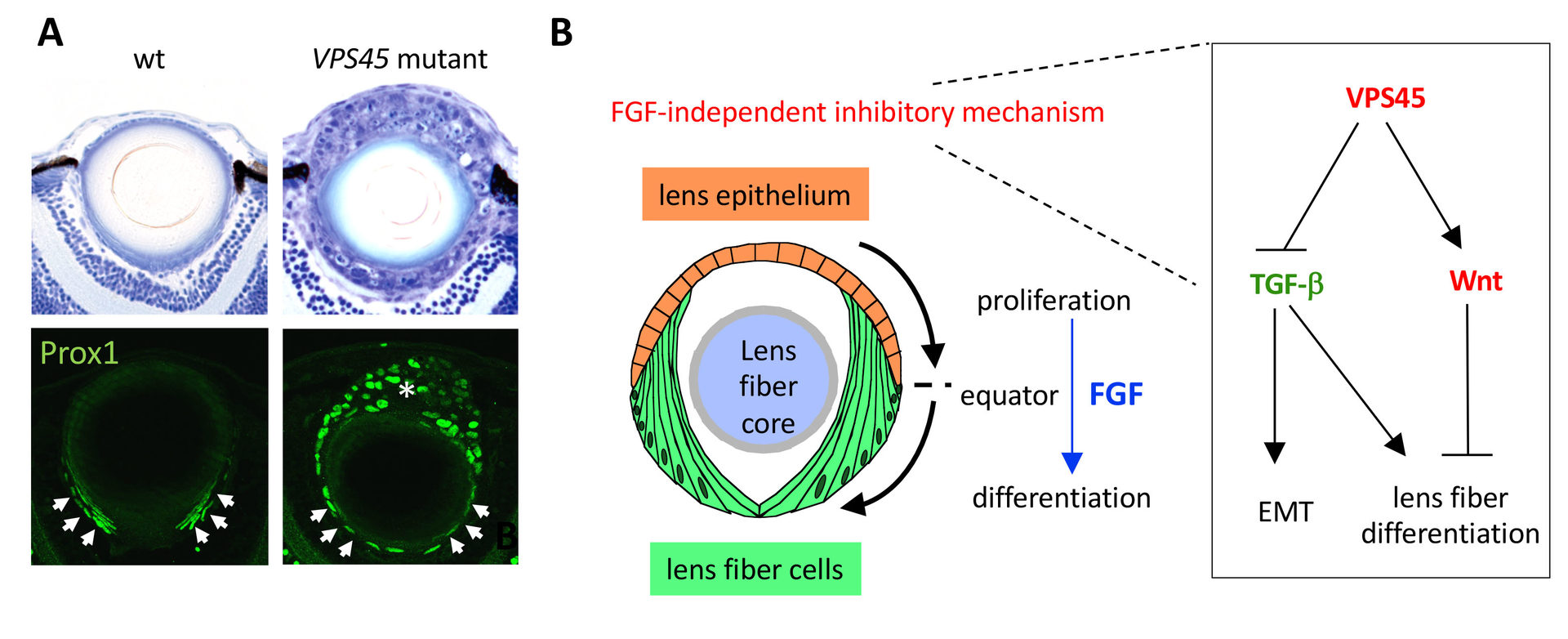
In addition, we recently found that one of Fgfrl1 genes, Fgfrl1b, is specifically expressed in lens epithelium. FGF receptor-like 1 (Fgfrl1) has three extracellular immunoglobulin domains, which bind to FGF ligands, but lacks an intracellular kinase domain. In mammals, proteolytic cleavage of Fgfrl1 releases its ectodomain, which subsequently binds FGF ligands, suggesting that Fgfrl1 functions as a decoy receptor. To inhibit the function of Fgfrl1b, we injected its morpholino antisense into wild-type embryos and examined lens fiber cell differentiation. In Fgfrl1b morphant, expression of an FGF target was elevated, suggesting that Fgfrl1b suppresses FGF signaling. Consistently, the expression of a lens fiber differentiation marker was enhanced. Ectopic differentiation also occurred in lens epithelium of Fgfrl1b morphant. Thus, Fgfrl1b suppresses FGF signaling to ensure equator-specific onset of lens fiber differentiation.
4. Publications
4.1 Journals
- *Iribarne, M., Hyde, D. R. and Masai, I. (2019) TNFa induces Müller glia to transition from non-proliferative gliosis to a regenerative response in mutant zebrafish presenting chronic photoreceptor degeneration. Front. Cell Dev. Biol. 7, 296. DOI:10.3389/fcell.2019.00296
- Kinoshita, N., Huang, A., McHugh, T. J., Suzuki, S. C., Masai, I., Kim., I. H., Soderling, S. H., Miyawaki, A., and *Shimogori, T. (2019) Genetically Encoded Fluorescent Indicator GRAPHIC Delineates Intercellular Connections. iScience 15, 28-38.
- Kinoshita-Kawada, M., Hasegawa, H., Hongu, T., Yanagi, S., Kanaho, Y., Masai, I., Mishima, T., Chen, X., Tsuboi, Y., Rao, Y., *Yuasa-Kawada, J., and Wu, J. Y*. Explant Culture of the Embryonic Mouse Spinal Cord and Gene Transfer by ex vivo Electroporation. Bio-Protoc. 9, e3373. DOI:10.21769/BioProtoc.3373
4.2 Books and other one-time publications
Nothing to report
4.3 Oral and Poster Presentations
(Oral, International conference)
- Sugiyama, Y., and Masai, I. Live-imaging of collective migration of lens fiber cells, in ARVO annual meeting 2019, Vancouver, Canada, 28th April–2nd May 2019.
- Takeuchi, Y., and Masai, I. Decoy receptor, Fgfrl1, spatially regulates lens fiber differentiation through fine-tuning of FGF signaling, in 2019 International zebrafish Conference, Suzhou, China, 12th – 16th June 2019.
- Sugiyama, Y., and Masai, I. Live-imaging of collective migration of lens fiber cells, in 2019 International zebrafish Conference, Suzhou, China, 12th – 16th June 2019.
- Takeuchi, Y., Kojima, Y., and Masai, I. Decoy receptor, Fgfrl1, suppresses FGF signaling to ensure equator-specific onset of lens fiber differentiation, in International Conference on the Lens 2019, Kona, Hawaii, 8th-13th Dec 2019.
- Sugiyama, Y., and Masai, I. Cell rearrangement and shape change cooperatively drive lens cell movement to form a spherical tissue, in International Conference on the Lens 2019, Kona, Hawaii, 8th-13th Dec 2019.
(Poster, international conferences)
- Nishiwaki, Y., and Masai, I. ER-resident BH3-only protein, BNip1, is a safe guard that limits the upper threshold of vesicular transport, in ARVO annual meeting 2019, Vancouver, Canada, 28th April–2nd May 2019.
- Nishiwaki, Y., and Masai, I. ER-resident BH3-only protein, BNip1, is a safe-guard that limits the upper threshold of vesicular transport, in 2019 International zebrafish Conference, Suzhou, China, 12th – 16th June 2019.
(Invited talks)
- Masai, I. “Mechanism regulating lens fiber cell differentiation –Genetic analysis using zebrafish as a vertebrate animal model–”, Annual meeting of Japanese society of cataract research, at Wakayama-big-Ai, Wakayama, Japan, 19th –20th July 2019.
(Oral, domestic conferences)
- Ahmed, M., Kojima, Y. and Masai, I. Mutation in strip1 gene leads to impaired retinal neural circuit formation in zebrafish, in Annual Meeting of 52nd JSDB, Osaka, Japan, 14th – 17th May 2019.
- Takeuchi, Y., and Masai, I. Decoy receptor, Fgfrl1, spatially regulates lens fiber differentiation through fine-tuning of FGF signaling, in Annual Meeting of 52nd JSDB, Osaka, Japan, 14th – 17th May 2019.
(Poster, domestic conferences)
- Ahmed, M., Kojima, Y. and Masai, I. Mutation in strip1 gene leads to impaired retinal neural circuit formation in zebrafish, in Annual Meeting of 52nd JSDB, Osaka, Japan, 14th – 17th May 2019.
- Sugiyama, Y., and Masai, I. Cell rearrangement and shape change cooperatively drive lens cell movement to form a spherical tissue, in 42th Annual meeting of the Molecular Biology Society of Japan, Fukuoka, Japan, 3rd – 6th Dec 2019.
5. Intellectual Property Rights and Other Specific Achievemen
5.1 Funding
- HFSP Research Grant
- PI name: Greg Stephens (Vrije Universiteit Amsterdam)
- Co-PI name: Joshua Shaevitz (Princeton Univ)
- Co-PI name: Ichiro Masai (OIST)
- 2016-2018
6. Meetings and Events
6.1 OIST Seminar
- Date: 22 July 2019
- Venue: OIST Campus Lab1
- Speaker: Keisuke Yonehara, Group Leader/Associate Professor (DANDRITE, Nordic EMBL, Dept. of Biomedicine, Aarhus University)
- Title: Visual motion processing from retina to visual cortical areas in mice
6.2 OIST workshop
- Title: Retina - Mechanism of photoreceptor degeneration and regeneration, and roles of immune system
- Date: 11-15 November 2019
- Venue: OIST Campus Lab1
- Co-organizers: Sumiko Watanabe (University of Tokyo), Akira Murakami (Juntendo University)
- Speakers:
- Dusanka Deretic (UNM school of Medicine)
- Silvia Finnemann (Fordham University)
- Seth Blackshaw (Johns Hopkins University)
- Daniel Goldman (Michigan University)
- David Hyde (University of Notre Dame)
- Brian Perkins (Cleveland Clinic)
- Deepak Lamba (UCSF)
- Kazu Kikuchi (National Cerebral Cardiovascular Center Res. Inst)
- Jeff Mumm (John Hopkins University)
- Wai Wong (NIH)
- Tian Xue (Univ. Sci. Tech. Chia)
- Jin Woo Kim (KAIST)
- Takahisa Furukawa (Osaka University)
- Ichiro Masai (OIST)
- Sumiko Watanabe (University of Tokyo)
6.2 OIST course
- Title: Developmental Neurobiology Course 2019
- Date: 30 July – 11 August 2019
- Venue: OIST campus Lab1
- Co-organizers: Yoko Yazaki-Sugiyama (OIST), David L. van Vactor (Harvard Medical School, OIST), Hiroshi Kawasaki (Kanazawa University), Hiroshi Kohsaka (University of Tokyo)
- Speakers:
- Rafael Yuste (Columbia)
- Naoshige Uchida (Harvard)
- Joshua Johansen (RIKEN)
- Yang Dan (California Berkeley)
- Takeshi Imai (Kyusyu Univ)
- Wen-Biao Gan (New York University School of Medicine)
- Jesse H. Goldberg (Cornell)
- Yutaka Yoshida (OIST)
- Stephanie L. Gupton (University of North Carolina)
- Michisuke Yuzaki (Keio)
- Kristin White (MIT/Harvard)
- Filippo Del Bene (Institut Curie)
- Akinao Nose (Tokyo Univ)
- Paul Garrity (Brandeis)
- Ikue Mori (Nagoya Univ)
- Ichiro Masai (OIST)
- Yoko Sugiyama (OIST)
- David van Vactor (OIST/Harvard)
- Tutors:
- Jelena Katic (OIST)
- Takashi Kudo (OIST)
- Anna Kuneji (Shinjo) (OIST)
- Yuichi Morohashi (OIST)
- Yuko Nishiwaki (OIST)
- Yuki Sugiyama (OIST)
- Yuki Takeuchi (OIST)
7. Other
Nothing to report.